A recipe for scalable quantum dots
A team including researchers from the Department of Physics at ETH Zurich found a method for creating electrically defined quantum dots with the potential for long-sought tunability and scalability.
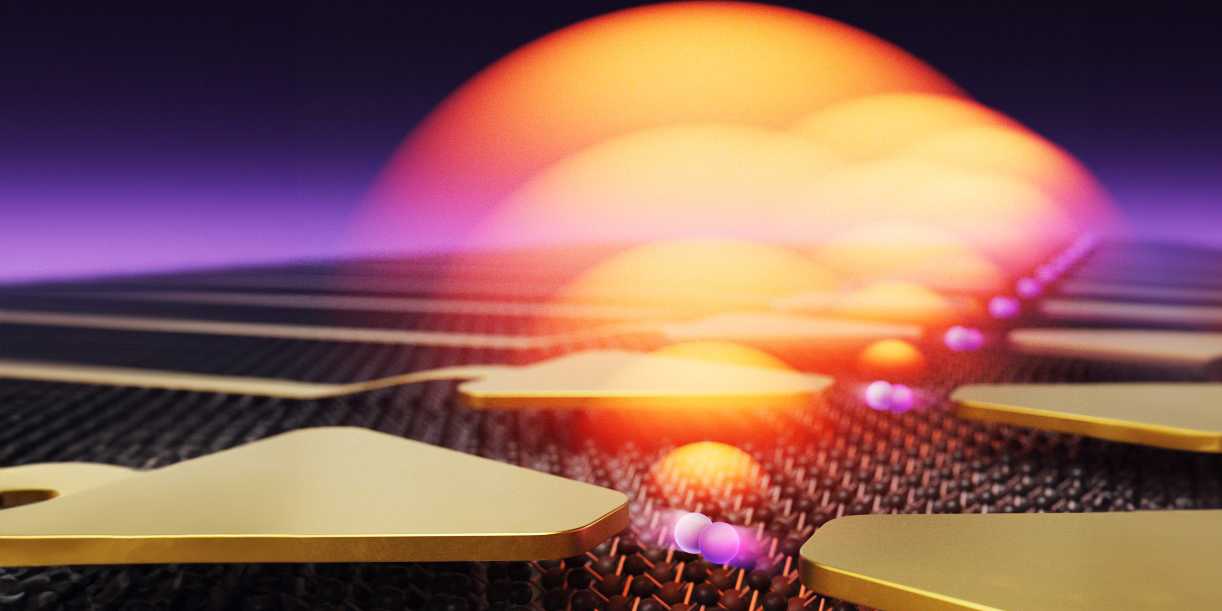
Quantum dots are nanoscale semiconductor structures with unique properties that hold promise for potential applications in areas such as display technology, photovoltaics and quantum computing. However, traditional methods for creating quantum dots – which rely on chemical synthesis or material epitaxial growth – impact the prospect of scalability for these structures. Another challenge with quantum dots is particularly relevant for photonic quantum computing applications: quantum dots are known to be excellent single-photon sources, but photons don't naturally interact with one another in the way that would normally be required for information processing. Promising mediators of photon-photon interactions are excitons, bound electron-hole pairs that play a crucial role in light-matter interactions. Achieving precise and scalable control over excitons confined in semiconductor heterostructures such as quantum dots has been a long-standing issue in solid-state optics.
A team of researchers led by Professor Puneet Murthy at ETH Zurich and Dr Thibault Chervy at NTT Research, Inc. Physics & Informatics Laboratories in the US has now reported successful quantum control of excitons in two-dimensional semiconductors. In an article just published in the journal Science Advances, the researchers describe how they confined excitons in various geometries, including quantum dots and quantum rings, by applying electric fields through lithographically nanostructured gate electrodes to control the motion of excitons in a monolayer transition metal dichalcogenide semiconductor. Thanks to spectroscopic tools they were able to observe discrete states of excitons, which is a signature of quantum confinement. "With conventional methods, quantum dots end up at random positions and have a broad distribution of energies. We showed how to create quantum dots by placing bow-tie-shaped electrodes close to a semiconductor material," says Murthy, whose Nanoscale Quantum Optics group is part of the Institute for Quantum Electronics. The team could not only define where to produce the desired quantum-dot-like confinement for the excitons but also demonstrate wavelength tunability of the quantum dots by varying the voltage applied on the electrodes. This approach is incredibly versatile too: modifying the structure of the gate electrodes allowed the researchers to realise different confinement geometries, the most relevant being quantum dots and rings.
The work of Murthy and co-authors further addresses the issue of scalability by showing how setting the right voltage on each electrode in an array of quantum emitters makes it possible to bring multiple quantum dots to the same energy, which is a highly relevant capability for future applications. "For photonic quantum computing we need an architecture that can scale up to thousands of identical quantum dots that act as single-photon sources. This is why electrical control is so important," says Chervy. "Our architecture is not different in nature from a transistor – after all, we keep a well-defined voltage potential across a tiny little junction."
The researchers believe that their work opens new research directions, both fundamental and applied. Thinking ahead, for example, Murthy envisages an array of a hundred identical quantum-dot sources, placed in an optical cavity for emission control. This could induce strong interactions between photons, giving access to the regime of quantum many-body photonics and facilitating the search for elusive collective photonic phases.
This news article is based on external page the press release issued by NTT Research, Inc. and on external page the blog article written by Professor Murthy and Dr Chervy for the NTT Research, Inc. website.
Reference
Hu, J. et al. Quantum control of exciton wave functions in 2D semiconductors. Sci. Adv. 10, eadk6369 (2024). external page DOI:10.1126/sciadv.adk6369